Mass spectrometry is potentially a powerful alternative to radioactive counting in the determination of cosmogenic nuclides because it utilizes every atom of this nuclide in the sample. In contrast, counting determinations utilize only the small number of atoms that decay during the measurement experiment. If decay rates are very high (corresponding to half-lives of less than a thousand years), then counting may be most efficient. However, for longer-lived nuclides, mass spectrometry has the ability to outperform counting.
Cosmogenic nuclides are characterized by very low abundances, both relative to other isotopes of the same element and to isobaric interferences from other elements. The first problem is exemplified by the fact that even modern carbon, with the highest 14C/12C ratio (1.2 H 10!12), would yield a 14C peal too small to see above the tail of the very large 12C peak in the mass spectrum of a ‘conventional’ mass spectrometer used by geologists. Such machines typically have ‘abundance sensitivities’ (peak tail at one mass unit distance) of 10!6 at the uranium mass, which may decrease to ca. 10!9 at the mass of carbon.
Abundance sensitivity might be improved sufficiently to measure 14C in a ‘conventional’ mass spectrometer by increasing the magnet radius, introducing electrostatic filters, and increasing the accelerating potential and magnet current. The latter approaches respectively filter and overwhelm the spread of energies of the ions emitted by the source. Accelerator mass spectrometers usually have all of these features (Fig. 14.20), but they are not central to accelerator mass spectrometry (AMS). In contrast, the three principle attributes of the tandem accelerators used in AMS are the positive ion source, the charge-exchange process, and the very high ion energies achieved, which allow the use of special detectors.
Lal (1988) suggested that the principal impetus for the development of AMS was the fact that accelerators became available for this purpose as their applications in physics diminished. As with many techniques, AMS began as a method looking for an application but quickly took off as a useful tool in its own right. An alternative method of excluding isobaric interferences is laser-induced resonance ionization (e.g., Labrie and Reid, 1981). However, this method has not lived up to its early promise.
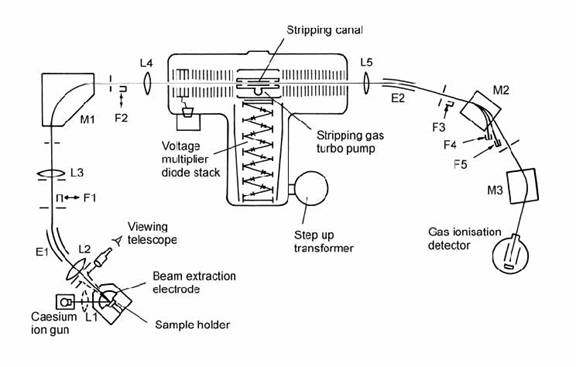
Fig. 14.20. Schematic illustration of the Toronto tandem accelerator showing typical features of such instruments. M1 ) M3: magnetic analysers; E1 ) E2: electrostatic analysers; L1 ) L5: electrostatic lenses. F1 ) F5: Faraday cups; After Kieser et al. (1986).
Check out the first part here.
The essence of the tandem accelerator is the initial acceleration of negative ions by a positive potential in the mega-volt range, followed by charge exchange of the ion beam, after which positive ions are accelerated back to zero potential. During the charge-stripping process, isobars of different elements often adopt different charge states, allowing their subsequent separation, while molecular ion isobaric interferences are destroyed.
Charge stripping may be performed by passing the ion beam through an electron-stripping gas (e.g., argon), through a thin graphite film, or (in very high energy accelerators) a thin metal foil. Experience with carbon has shown that charge stripping to a 3+ state is often most effective since CH2 (the principal molecular ion interference) breaks apart rather than forming triple-charged ions (Litherland, 1987). This avoids the need for a high-resolution magnetic analyzer to resolve molecular ions by their mass defect. By using only a low-resolution magnetic analyzer, the instrument’s transmission for rare isotopes (e.g., 14C) is maximized.
Radiocarbon Dating by AMS
Most 14C analyses by AMS are presently performed on solid graphite samples. A typical preparation method is the catalytic reduction of CO2. In order to achieve a 14C ion beam of 15 ions per second from modern carbon, an ‘intense’ 12C ion beam of 2:A must be generated. This is normally achieved using a cesium sputter source, which ejects negative carbon ions by bombarding the sample with Cs+ from an ion gun. The efficiency of AMS radiocarbon measurement is illustrated by the fact that it yields the same count rate from 1 mg of carbon as the $ count rate from a whole gram of carbon. Nevertheless, a 55-year-old carbon sample yielding a 2:A 12C beam still has a 14C count rate of only one ion per minute (corresponding to a 14C/12C ratio of ca. 1.2 H 10!15).
The determination of 14C (and 26Al and 129I) can be performed on a ‘low-energy’ tandem accelerator (Litherland, 1980; 1987), because the direct atomic isobars of these species (14N, 26Mg and 129Xe) do not form stable negative ions. Therefore, complete separation from these species occurs in the source (e.g. Purser et al., 1977). However, the separation of the atomic 14C! Ion from the molecular ion 12CH2! Depends on the charge-stripping stage of the tandem accelerator.
The sputter source generates an ion beam with variable ion energies. After acceleration to a few tens of kilovolts, this beam must be ‘cleaned up’ using an electrostatic analyzer before the beam is ready for the accelerator. In addition, it is necessary to split the major and minor ion beams with a magnetic analyzer before the accelerator in order to minimize the scattering of the 14C beam by the collision of the 12C beam with gas molecules.
In 14C dating, the most effective charge-stripping medium is provided by a relatively higher gas pressure in the central ultra-high-voltage ‘stripping canal’ of the tandem accelerator (Fig. 14.20). Differential pumping of the acceleration tubes at either end of the tandem generator can maintain a pressure 5000 times lower here than in the stripping canal (Litherland, 1987). The charge-stripping process generates a range of charge states in the positive ion beam, such that only ca. 50% of ions have the selected charge. Therefore, the accelerator system must be calibrated against standards of known 14C/12C ratio before unknown samples are run. Production of 14C3+ using a 3 MV accelerator is ideal for radiocarbon measurement, but 14C2+ ions from a 1.4 MV accelerator can also be used (Lee et al., 1984).
The very high energy of the positive ion beam at the collector end of the instrument (normally > 1 MeV) allows the use of ionization counters which can identify collected ions as well as measure their abundance. This is done by measuring the energy loss of the ions in a ‘collision cell’, in which the ion beam is gradually decelerated by collision with gas molecules. Different kinds of ions lose energy at different rates in the collision cell. Hence, this provides a final means of resolving any residual 12C–molecular ions in the 14C beam (possibly generated by recombination after the accelerator). Fig. 14.21 shows that the molecular ion beams of 13C and 12C are barely significant in modern carbon but dominant in 47 kyr-old carbon.
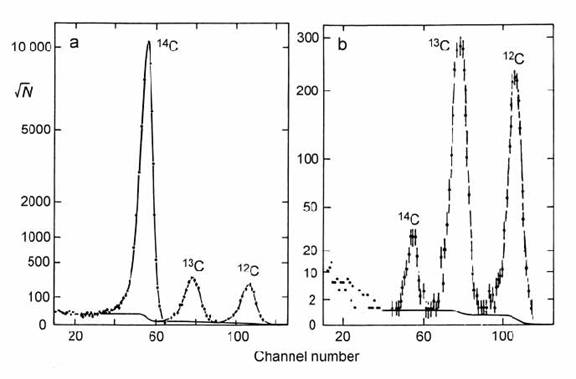
Fig. 14.21. Multi-channel pulse-height (energy-loss) analysis of radiocarbon dating samples from an ionization detector. a) Modern carbon; b) 47.4 kyr-old carbon. Typical error bars are shown. After Litherland (1987).
A good example of the application of AMS to radiocarbon analysis is provided by the dating of the Shroud of Turin (Damon et al., 1989). This was believed to be possibly the burial cloth of Christ, although its sudden appearance in the 1350s raised the probability that it was instead a medieval ‘icon’. The advent of AMS analysis provided the opportunity to perform an absolute date on the fabric of the shroud, using a total of only 7 cm2 (150 mg) of cloth. This was divided between university laboratories in Arizona, Oxford, and Zurich. A major worry was that the fabric might have been contaminated during its long history as a relic. Therefore, each group performed a variety of different cleaning procedures on different sub-samples of cloth. In addition, each group dated three control fabric samples of different known ages to form a general impression of the between-lab reproducibility.
The results from the three laboratories collated independently at the British Museum were in good agreement, although the shroud itself yielded poorer reproducibility than the controls, possibly due to contamination. The ‘conventional’ radiocarbon age of the shroud (B.P.) was translated into a calendar age using the calibration curve of Stuiver and Pearson (1986). The 95% confidence limits on the conventional age were found to bracket an inflection in the calibration curve, which technically creates two possible calendar age ranges, A.D. 1262)1312, and 1353)1384 (Fig. 14, .22). However, since the shroud went on view in the 1350s, the latter range can, fortunately, be excluded on historical grounds. It is concluded that the linen of the shroud was derived from cotton, which grew in A.D. 1290 ” 25 years ago, and it is, therefore, a medieval artifact.
Fig. 14.22. Translation of the ‘conventional’ radiocarbon age for the Shroud of Turin into a calendar age. Age limits are at the 95% confidence level (2F). Of the two possible calendar age ranges (shaded), the more recent is excluded by historical data. After Damon et al. (1989).
REFERENCES
Adkins, J. F. and Boyle, E. A. (1997). Changing atmospheric ) 14C and the record of deep water paleoventilation ages. Paleoceanography 12, 337–44.
Anderson, E. C. and Libby, W. F. (1951). World-wide distribution of natural radiocarbon. Phys. Rev. 81, 64)9.
Andree, M., Oeschger, H., Broecker, W., Beaven, N., Klas, M., Bonani, G., Hoffman, H. J., Suter, M., Woelfli, W. and Peng, T.-H. (1986). Limits on ventilation rates for the deep ocean over the last 12,000 years. Climate Dynamics 1, 53–62.
Andrews, J. N., Davis, S. N., Fabryka-Martin, J., Fontes, J. C., Lehmann, B. E., Loosli, H. H., Michelot, J-L., Moser, H., Smith, B. and Wolf, M. (1989). The in situ production of radioisotopes in rock matrices with particular reference to the Stripa granite. Geochim. Cosmochim. Acta 53, 1803)15.
Arnold, J. R. (1956). Beryllium-10 produced by cosmic rays. Science 124, 584)5.
Arnold, J. R. (1958). Trace elements and transport rates in the ocean. 2nd UN Conf. on Peaceful Uses of Atomic Energy 18, 344)6. IAEA.
A